In the high-confinement regime of
tokamak plasmas---essential to achieve a burning
plasma---a transport barrier forms at the edge of
the plasma, believed to be caused by suppression of
ion-scale turbulence by pressure-gradient-driven
sheared flows. The physics of the resulting edge
"pedestal" region sets the confinement properties of
the whole plasma and is thus pivotal for the entire
device. The pedestal is a complex nonlinear system
characterised by an interplay between steep
equilibrium gradients (of density and temperature)
and the turbulence that they trigger [1,2,3]---the
turbulent particle and heat fluxes caused by this
turbulence in turn decide the size of the gradients.
A critical electron temperature gradient,
proportional to the density gradient [4], is
required for the linear instability [5] that drives
electron-scale ETG turbulence and hence a finite
heat flux. The formation of the electron-temperature
pedestal is therefore intimately related to that of
the density pedestal. Nonlinear gyrokinetic
simulations [6,7] show that at significantly steeper
gradients than the linear threshold, above the
experimental operating point, the heat flux
increases faster than linearly with the driving
gradient, i.e., transport becomes "stiff", clamping
the profiles to this nonlinear threshold. The
existence of this threshold is thought to be related
to the appearance of modes with a high parallel
wavenumber, which are resonant with the parallel
electron motion. This project's aim is to sort out
the fundamental physics behind these phenomena and
hence their quantitative dependence on the
equilibrium parameters. Detailed comparisons of the
outcome with experimental pedestal profile data from
JET-ILW and MAST-U can be made over a range of
conditions. Such information, suitably
parameterised, can then be used to develop a reduced
model of the pedestal, which is required to design
future burning-plasma devices, e.g., STEP.
|
|
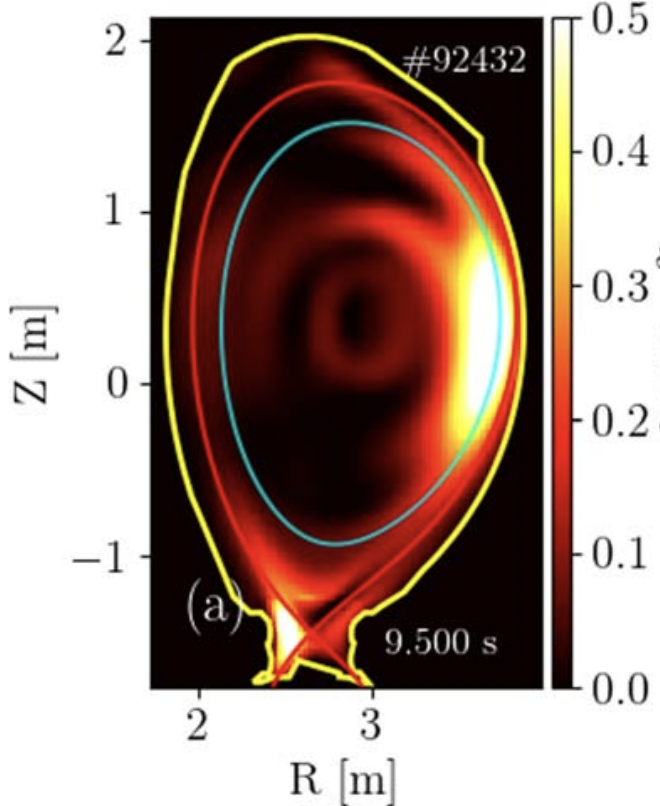
|